FISHing for pathogens: Rapid detection of whole microbial cells in foods
- Like
- Digg
- Del
- Tumblr
- VKontakte
- Buffer
- Love This
- Odnoklassniki
- Meneame
- Blogger
- Amazon
- Yahoo Mail
- Gmail
- AOL
- Newsvine
- HackerNews
- Evernote
- MySpace
- Mail.ru
- Viadeo
- Line
- Comments
- Yummly
- SMS
- Viber
- Telegram
- Subscribe
- Skype
- Facebook Messenger
- Kakao
- LiveJournal
- Yammer
- Edgar
- Fintel
- Mix
- Instapaper
- Copy Link
Posted: 30 June 2010 | Byron Brehm-Stecher, Assistant Professor, Rapid Microbial Detection and Control Laboratory, Iowa State University | No comments yet
Today’s food production and distribution networks are extremely efficient. We are able to move food from the field to the table rapidly and effectively – on a global scale – under conditions that maximise quality, visual appeal and nutritional content. Unfortunately, toxigenic or infectious microbes may also come along for the ride at any number of points along this farm-to-fork journey. As we have seen with recent incidents in the United States, contaminated ingredients provided to large food companies by relatively small niche players can wreak havoc throughout the food chain.
Today’s food production and distribution networks are extremely efficient. We are able to move food from the field to the table rapidly and effectively – on a global scale – under conditions that maximise quality, visual appeal and nutritional content. Unfortunately, toxigenic or infectious microbes may also come along for the ride at any number of points along this farm-to-fork journey. As we have seen with recent incidents in the United States, contaminated ingredients provided to large food companies by relatively small niche players can wreak havoc throughout the food chain.
Today’s food production and distribution networks are extremely efficient. We are able to move food from the field to the table rapidly and effectively – on a global scale – under conditions that maximise quality, visual appeal and nutritional content. Unfortunately, toxigenic or infectious microbes may also come along for the ride at any number of points along this farm-to-fork journey. As we have seen with recent incidents in the United States, contaminated ingredients provided to large food companies by relatively small niche players can wreak havoc throughout the food chain.
Examples include the nut paste and other peanut products sold by the Peanut Corporation of America, the crushed black pepper added to over one million pounds of processed meat or the hydrolysed vegetable protein produced by one small Nevada firm, all of which were recalled due to contamination with Salmonella. Because these materials were used as base ingredients in foods produced by many other firms, their contamination with Salmonella echoed disproportionately throughout the food chain, leading to thousands of product recalls, hundreds of illnesses and in some cases, death.
Table 1 lists key governmental food recall alerting services serving the United States, the UK or the European Union. Simple searches of these databases serve as ready reminders of how susceptible our food system is to contamination with microbial pathogens.
Table 1. Food Recall Alerting Services
Food Recall Alerting Services | URL |
FSIS Recalls & Alerts (United States Department of Agriculture Food Safety and Inspection Service) | http://www.fsis.usda.gov/fsis_recalls/Open_Federal_Cases/ |
Food Alerts (United Kingdom Food Standards Agency) | http://www.food.gov.uk/enforcement/alerts/ |
RASFF Portal (European Union Rapid Alert System for Food and Feed) | http://ec.europa.eu/food/food/rapidalert/rasff_portal_database_en.htm |
Limits of culture-based testing: the need for rapid methods
Traditional, culture-based methods for detection and enumeration of microorganisms require relatively long periods to yield results – maybe as long as a week for some pathogens. If traditional methods for detection of pathogens are to be used, today’s food processors have two options to monitor and help ensure the safety of their products. They can either hold their product on site until testing results are ready, or they can ship the food, hoping that it’s safe. Unfortunately, holding foods on site can be costly, especially for refrigerated or other ‘high maintenance’ foods, and reduces the amount of time the product is available for purchase before it spoils. Foods that have already been shipped can always be recalled if traditional testing indicates the presence of a pathogen – provided they haven’t already been purchased and consumed.
The disconnect between how quickly we are able to produce and globally distribute foods and the relatively slow pace of traditional culture-based microbiology highlights the need for rapid, alternative methods for detecting pathogens in foods. A number of rapid methods have been developed over the years, ranging from relatively simple tests such as lateral flow (‘dipstick’) assays, to more complex immunological or molecular tests, such as automated Enzyme-Linked Immunosorbent Assay (ELISA) or the Polymerase Chain Reaction (PCR). Intense interest in faster testing for pathogens has also spurred the development of a constellation of media-based products, including novel chromogenic agars, enrichment broths containing time-release selective agents or smaller, rehydratable versions of traditional media designed to compact and simplify microbial testing.
The ‘gold standard’, advantages of cell-based methods
The methods we use to grow microbes have remained essentially unchanged for over 100 years and culture-based methods are still regarded as the ‘gold standard’ for detection and characterisation in both food and clinical microbiology. This stems from both the long, proven track record for cultural techniques and from their relative simplicity and ease of use. Using cultural methods, we can amplify (enrich) small numbers of target cells present as contaminants in our foods. After culture, we can subject isolates to further testing, including morphological and biochemical characterisation. In the last 20 years, there has been an explosion in molecular techniques for rapid detection and characterisation of micro – organisms. Still, many molecular tests used today are not fully culture-independent, and often include a cultural step such as nonselective pre-enrichment.
Hardcore proponents of molecular microbiology might suggest that the primary reason food microbiologists are still so reliant on cultural techniques is that we are too conservative and suffer from a resistance to change. It is true that food microbiologists may not be early adopters of new technologies to the same degree as clinical microbiologists, but this is partly due to economic drivers, with cost-per-test being less of a barrier in the clinic than in the testing of low-margin or commodity foods. However, another important reason that we are reluctant to let go of culture-based approaches is that they enable us to work directly with the cells, rather than with disembodied cellular components such as the enzymes, antigens, nucleic acids or other pathogen-derived components detected using other methods. After all, apart from toxins, it is the cells that cause disease, so it is both natural and relevant for us to think and operate within a cellular context. At the very least, food micro biologists need to answer the following questions:
1. Are there pathogenic cells in the food (presence/absence)?
2. If so, how many are present (quantitation/enumeration)?
Table 2. Commercial FISH Kit Suppliers
Company | Product Name | Description | Website |
AdvanDx, Inc. (Woburn, MA, USA) | PNA FISH™ | Peptide nucleic acid (PNA)- based probe kits for detection of clinical pathogens | http://www.advandx.com |
BioVisible BV (Groningen, NL) | FISH-kit™, SepCheck™ | DNA-based probe kits for detection of bacteria (food, environmental, clinical) | http://www.biovisible.com/ |
Microgen Bioproducts Ltd.
(Surrey, UK) |
BACTfish™ | DNA-based probe kits for clinical pathogens (Available in UK only) | http://www.microgenbioproducts.com/ |
Vermicon AG (Munich, DE) | VIT® | DNA-based probe kits for detection of bacteria (food, environmental, clinical) | http://www.vermicon.com/en/en/index.html |
Fluorescence in situ hybridisation for molecular detection of whole cells
Fluorescence in situ hybridisation (FISH) is a whole cell method for molecular detection of target microbes, first described in 1989 by De Long and colleagues7. As a whole cell method, FISH can provide diagnostically important cellular information that is not available outside the context of the cell, including information on cell morphology or cell number and distribution within a sample. In some cases, FISH can also be used to correlate cell type (genetic identity) with biochemical activities. In food or clinical microbiology, FISH can be used to rapidly label specific cell types, allowing their detection and enumeration in complex samples via fluorescence microscopy or flow cytometry.
In the FISH technique, fluorescently-labelled nucleic acid probes are directed to complementary rRNA targets located on ribosomes inside intact cells. Natural sequence variation in 16S or 23S rRNA among microbes can be used to create probes recognising microbial cells at the genus, species and in some cases, subspecies levels. Because FISH allows staining of specific cell types according to evolutionary differences in RNA sequence, it has also been aptly described as a ‘phylogenetic stain’. A model of the 23S ribosomal subunit highlighting the locations of selected universal, genus or species-specific probe binding sites is shown in Figure 1.
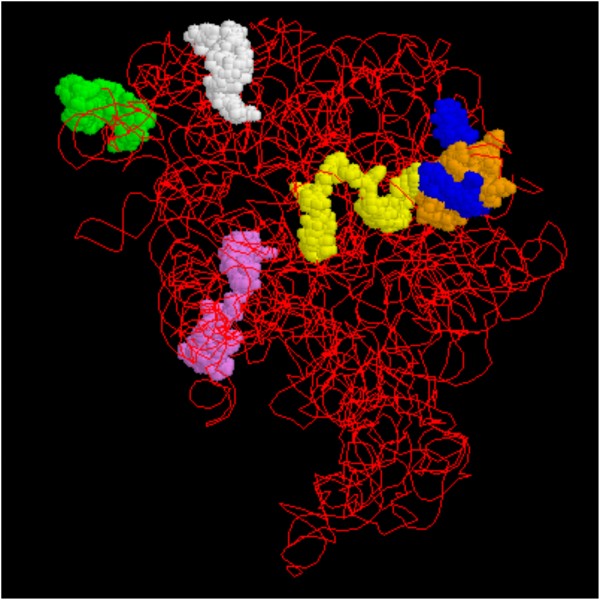
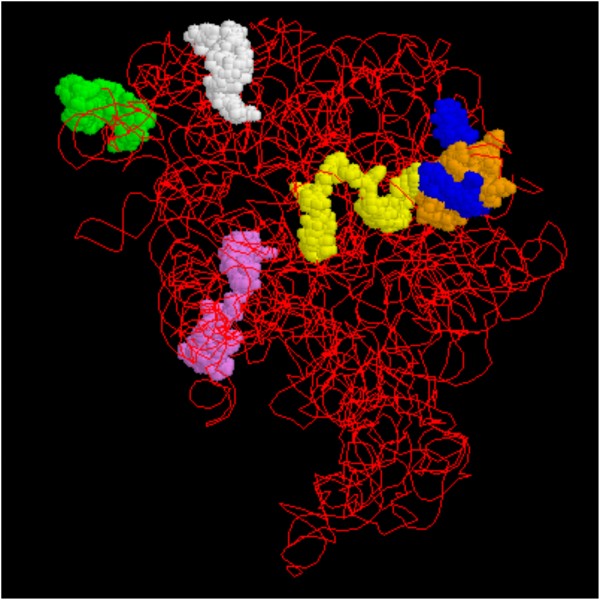
Figure 1 Model of the 23S ribosomal subunit. Binding sites for probes sourced from the literature are shown. Probe specificities and binding positions (E. coli numbering) are given below. Clockwise from upper left, they are: • Green: Salmonella, positions 1713-17301 • White: Pseudomonas spp., positions 1432-14462 • Blue: Vibrio vulnificus, positions 287-3042 • Orange: S. aureus, positions 327-3493 • Yellow: Lactococcus lactis, positions 271-2892 (overlaps with V. vulnificus) • Purple: Bacteria (Universal), positions 1933-19512
The steps used in FISH probe development are summarised in Figure 2, using a Salmonella-specific FISH probe as an example. Briefly, rRNA gene sequences for both target and closely related non-target cells are aligned and compared. One or more short (~15-30 base) regions where target and non-target cell sequences differ are chosen for exploration as possible probe sequences. For very closely related cell types, sequence differences may be minimal and cross-hybridisation may occur. Probe sequences containing central mismatches are typically the most discriminatory. Several versions of each probe may need to be examined experimentally in order to identify the most suitable sequences.
Next, candidate probes are synthesised and examined experimentally for their abilities to hybridise to whole permeabilised cells. It is important to note that even if the primary (linear) rRNA gene sequence is variable enough to design a potentially useful probe, higher order structures of the ribosome (folding, knotting, binding of proteins) may limit access of probes to their targets in whole cells. Although in silico (database) screening can provide a broad idea of how specific a given probe may be for the target organism, in silico screening must be combined with empirical screening against panels of target organisms (to measure probe inclusivity) and non-target organisms (to measure probe exclusivity). Internet-based tools available for checking the in silico specificity of probes against curated sequence databases include probecheck (http://131.130.66.200/cgi-bin/probecheck/probecheck.pl), which allows matching against both large and small subunit databases, and Probe Match (http://rdp.cme.msu.edu/probematch/search.jsp), which checks sequences against a 16S rRNA database.
‘Shake and bake’ labelling of target cells
The hybridisation process is relatively straightforward and can be streamlined so that it is basically a ‘shake and bake’ process no more challenging than standard antibody-based staining. An overview of the FISH process is shown in Figure 3. For liquid samples or broth cultures, the first step in the FISH process is harvesting of the cells, which can be done using either filtration or centrifugation. For more complex samples, additional sample preparation methods may be needed, depending on the sample type. Examples include treatment of full fat milk with chelators and detergents, followed by centrifugation. These steps are used to lyse somatic cells, remove fat and pellet bacteria prior to analysis.
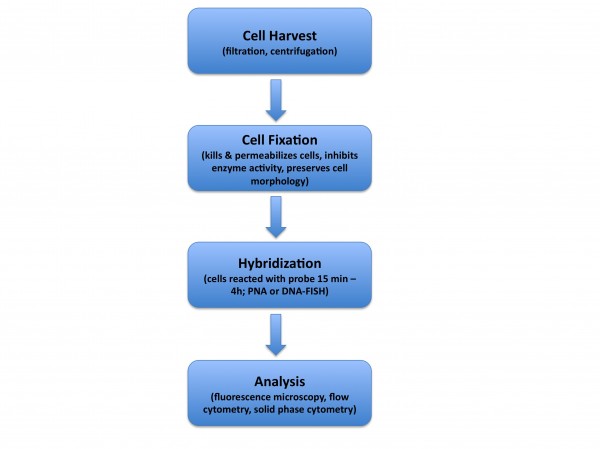
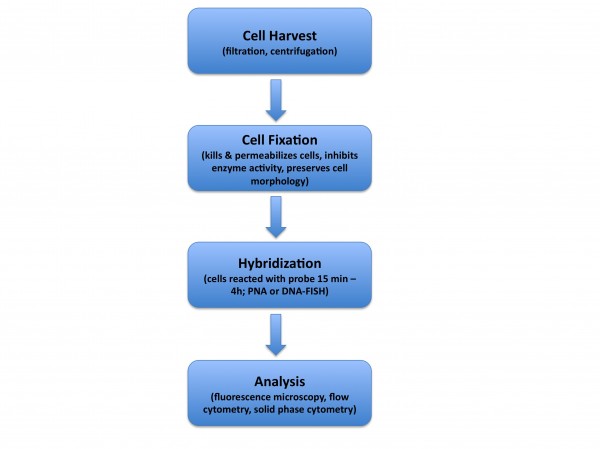
Figure 3 Overview of the FISH process for staining whole microbial cells. Cells are harvested via filtration or centrifugation, permeabilised with fixative, reacted with the probe and analysed via fluorescence microscopy or flow cytometry.
The next step is cell fixation, which is typically carried out using either aldehyde or alcohol fixatives (formalin or ethanol, for example). The goals of the fixation step are to permeabilise target cells to FISH probes, to inhibit enzyme activity (e.g. RNases and DNases), to retain target molecules within the cell and to preserve cell morphology. Fixation will also kill pathogens, providing an additional measure of safety during downstream sample handling and analysis. Fixative is removed and cells are hybridised with a probe (or a mixture of probes) by resuspending the sample in a buffered solution containing probe, salt (sodium chloride) and detergent (sodium dodecyl sulphate) and heating this mixture for a sufficient period of time to allow bright cell labelling. The time needed for adequate cell labelling ranges from minutes to hours and is dependent on a number of variables, including probe concentration and hybridisation temperature, degree of cell permeabilisation and whether DNA or other types of probes are used (see below). Typically, excess probe is removed via washing, although in many cases a simple dilution step may be substituted for a formal washing. Hybridisation results can then be monitored using fluorescence microscopy or flow cytometry and labelled target cells are detected/enumerated.
Although a single FISH probe molecule will bind to just one target on the ribosome, active microbial cells typically contain thousands of ribosomes. This means that rRNA is a physically enriched (or amplified) target molecule. The aggregate signal of thousands of labelled probes binding to their complements on the ribosome leads to bright fluorescent labelling of target cells after FISH staining. Although some non-specific binding of probes to non-target cells can occur, steps can be taken to minimise this so that non-target cells are not labelled. An example of strong FISH probe staining, with multicolour staining for differentiation of Candida species, is shown in Figure 4.
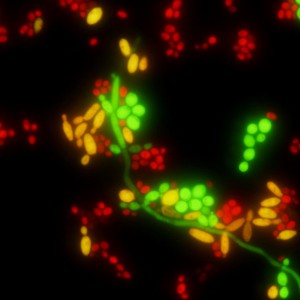
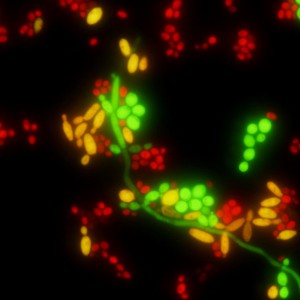
Figure 4 PNA FISH result for three Candida species using the Yeast Traffic Light™ kit from AdvanDx, Inc. (http://www.advandx.com). The kit uses a mixture of probes to provide colour-coded differentiation of yeast species within 90 minutes. In this mixed-species sample, C. albicans cells or pseudohyphae are green, C. tropicalis cells are yellow and C. glabrata cells are red. Copyright: M. Fiandaca, AdvanDx, Inc.
Peptide Nucleic Acids
Although DNA-based probes are the most commonly used, other probe chemistries are available, including peptide nucleic acid (PNA) probes. PNAs were first described by Nielsen and colleagues in the early 1990’s and have a number of unique and valuable properties (http://www.saworldview.com/article/p-is-thenew- d). PNAs are synthetic DNA mimics made by attaching either natural or non-natural nucleobases onto a repeating backbone of amide-linked N-(2-aminoethyl) glycine units. As with DNA probes, PNAs hybridise to complementary DNA or RNA sequences through Watson-Crick base pairing, but their uncharged and hydrophobic backbones provide several advantageous properties over DNA-based probes. These include biological stability, faster hybridisation, high binding affinity for target nucleic acids and the ability to penetrate ‘difficult’ biological structures (thick bacterial cell walls, parasite cysts, etc.). An additional advantage of PNA probes is their ability to bind to portions of the ribosome that are physically inaccessible to traditional DNA probes. This stems from the fact that PNAs are hybridised under low salt (≤100 mM NaCl), high pH (9.0) high temperature (55°C) conditions, resulting in a denatured (‘loosened’ or ‘unravelled’) rRNA that probes can more easily penetrate and bind within. Foodborne bacteria for which PNA probes have been described include Listeria spp., Salmonella, Enterobacter (Cronobacter) sakazakii6 and Staphylococcus aureus. More background on PNA probes can be found here: http://www.advandx.com/technology/pna_fish.php.
Commercially available reagents
Although pathogen-specific FISH probes that are described in the scientific literature can easily be ordered from commercial oligo – nucleotide synthesis houses, commercial FISH probe kits are also available. These kits typically contain all the materials (probe, fixative, buffers, positive and negative control slides, etc.) and instructions needed for FISH-based analysis of samples. While some companies’ offerings are focused more on the clinical market, others supply kits for detection of various microbes (pathogens, quality indicators and spoilage organisms) that may also be present in environmental or food samples. Table 2 on page 15 lists some commercially available FISH kits, with associated web links. For those unfamiliar with FISH, use of commercial kits may hold special advantages apart from convenience, as these typically include access to customer or technical support in case problems are encountered.
Conclusions
As a means for rapid molecular labelling of whole target cells, FISH is an attractive means for screening foods for the presence of specific pathogens. Examples of how FISH has been used in the analysis of foods include ecological studies of artisanal cheese microflora, microscopic detection of Salmonella on fresh produce surfaces (tomatoes, spinach, jalapeño peppers)5 and detection of Campylobacter spp. in chicken livers8. The high specificity of FISH, combined with flow cytometric analysis has also proven to be a very effective approach for detection of Salmonella in alfalfa sprouts, a food that contains very high backgrounds (~108 CFU/ml) of closely-related non-target flora4. These studies demonstrate the robust and flexible nature of the FISH approach and highlight its utility as a valuable tool for whole cell molecular analysis of foodborne microbes.
References
1. Nordentoft, S., Christensen, H. and H.C. Wegener. 1997. Evaluation of a fluorescence-labelled olgonucleotide probe targeting 23S rRNA for in situ detection of Salmonella serovars in paraffin-embedded tissue sections and their rapid identification in bacterial smears. Journal of Clinical Microbiology 35: 2642-2648.
2. Amann, R.I., Ludwig, W. and K-H. Schleifer. 1995. Phylogenetic identification and in situ detection of individual microbial cells without cultivation. Microbiological Reviews 59: 143-169.
3. Veeh, R.H., Flood, J.A. and C.C. Davis. 2000. In situ identification of Staphylococcus aureus by whole-cell hybridization with fluorescently-labeled oligonucleotide probes. Abstract C-191, American Society for Microbiology’s General Meeting, Los Angeles, CA.
4. Bisha, B. and B.F. Brehm-Stecher. 2009. Flow-through imaging cytometry for characterization of Salmonella subpopulations in alfalfa sprouts, a microbiologically complex food system. Biotechnology Journal 4: 880-887.
5. Bisha, B. and B.F. Brehm-Stecher. 2009. Simple adhesive-tape-based sampling of tomato surfaces combined with rapid fluorescence in situ hybridization for Salmonella detection. Applied and Environmental Microbiology, 75: 1450-1455.
6. Brehm-Stecher, B.F., Hyldig-Nielsen, J.J., and E.A. Johnson. 2005. Design and evaluation of 16S rRNA- targeted peptide nucleic acid probes for whole cell detection of the genus Listeria. Applied and Environmental Microbiology 71: 5451-5457.
7. DeLong, E.F., Wickham, G.S., and N.R. Pace. 1989. Phylogenetic stains: ribosomal RNA-based probes for the identification of single cells. Science 243: 1360-1363.
8. Schmid, M.W., Lehner, A., Stephan, R., Schleifer, K.-H., and H. Meier. 2005. Development and application of oligonucleotide probes for in situ detection of thermotolerant Campylobacter in chicken faecal and liver samples. International Journal of Food Microbiology 105: 245-255.
About the Author
Byron Brehm-Stecher
Byron Brehm-Stecher received his PhD from the University of Wisconsin- Madison in 2002, where he studied rapid methods for the detection of foodborne pathogens. He came to Iowa State University in 2004 from a position as a senior scientist and molecular biologist with Applied Biosystems, Inc., Bedford, MA. He now heads the Brehm-Stecher Rapid Microbial Detection and Control Laboratory at Iowa State University.